Brio Functional Medicine
At Brio Functional Medicine, we are committed to helping you reach optimal health and vitality with cutting-edge, personalized medicine.
What Is Functional Medicine?
WHY BRIO FUNCTIONAL MEDICINE?
Brio Functional Medicine is Chattanooga, Tennessee and Northwest Georgia's comprehensive integrative medical provider blending cutting-edge science with the best of traditional treatment options. We are the referring provider for Dr. Scott Resnick of the former Chattanooga Functional Medicine. Brio is committed to treating the person and not simply their symptoms. We work to understand your history, your genetics, and your lifestyle to find the root cause of your condition. By doing so, we offer a more complete treatment experience with better health outcomes.
Founded by Meredith Mason, NP-C, Brio Functional Medicine provides patient-centered medical treatment and counseling to help patients take positive ownership of their health. When you are ready for a more comprehensive approach to medicine, you are ready for Brio. Contact us today to set up your initial appointment and let us show you the difference we can help you make.
WHY BRIO FUNCTIONAL MEDICINE?
The Brio Difference
Personal Relationships
We value our patients and their life experience
Quality Time
Extended one-on-one appointments with our provider
Comprehensive Treatments
Combining the best of traditional medicine with functional concepts
Patient Convenience
Short wait times and self-scheduling appointments
Collaborative Care
Patient preferences are considered in developing a personalized plan of care
Customer Service Commitment
We strive for an exceptional patient experience
Data-Driven
Data-driven diagnostics guide the treatment plan
Evidence Based Care
Bringing the newest science to the clinical setting
What Our Patients Say About Us
Resources
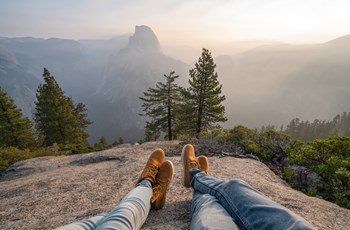
Anyone who has dealt with issues related to chronic fatigue has likely evaluated their hypothalamic–pituitary–adrenal (HPA) axis performance through a multi-point salivary test. Cortisol is readily measured in saliva when samples collected at predetermined intervals throughout a single day reveal one’s physiological resilience and metabolic reserve in response to daily stressors. HPA axis testing is a mainstay in the world of integrative, naturopathic, and functional medicine. For the past 18 months, the world has existed under the constant shadow of COVID-19. To say that we have been “stressed” is an understatement. Mental-emotional stressors certainly trigger the activation of the stress response pathways, but physiological and lifestyle factors contribute to these physiological processes as well. Furthermore, inflammation due to infection provoke the adrenal glands to release cortisol to regulate the inflammatory response and support the immune system. In the case of COVID-19, the research cited below has shown that cortisol does not rise in response to the SARS-CoV-2 infection as expected. In fact, surprisingly, the opposite is true - cortisol levels drop in the presence of SARS-CoV-2, leaving the body vulnerable to escalating and unchecked inflammation, and predisposing the individual to the life-threatening adrenal crisis. HPA axis dysfunction can occur under many types of chronic stressors; however, viral infections can trigger specific dysfunction within the HPA axis, leaving its host with chronic fatigue and weakness long after the initial infection has been resolved. Some of the latest research also takes a closer look at other endocrine organs such as the thyroid, hypothalamus, pituitary, pancreatic and gonadal tissue, and how each may be affected by the SARS-CoV-2 virus. In the last installment of this four-part series on long COVID, we will look at the mechanisms involved with the SARS-CoV-2 virus and the HPA axis, and what this means for COVID long-haulers. SARS-CoV-2 and Adrenal Insufficiency SARS-CoV-2 can influence cortisol output through three different pathways: In a letter to the editor of the American Journal of Physiology-Endocrinology and Metabolism, Siejka postulates that adrenal insufficiency (AI) related to COVID-19 may occur for the following reasons: 1) hypophysitis and adrenalitis resulting from direct infection with the SARS-CoV-2 virus, leading to secondary or primary AI; 2) production of adrenocorticotropic hormone (ACTH) antibodies; and 3) critical illness-related corticosteroid insufficiency (CIRCI) [1]. It has been determined that the hypothalamus, pituitary, and the adrenal glands all have angiotensin-converting enzyme (ACE)2 receptors so direct infection of these organs is plausible. In fact, histological findings report focal necrosis and vasculitis of the small veins of the adrenal glands, and structural and functional damage within the hypothalamus and the pituitary in those who were infected with the SARS-CoV-2 virus [1]. According to the recent article published in Medical Hypotheses, the uncanny ability of SARS-CoV-2 to inhibit the host’s corticosteroid stress response is a means to evade the immune system [2]. SARS viruses have a unique mechanism to inhibit the activation of the stress response and prevent cortisol release by expressing a series of amino acids that mimic parts of the ACTH amino acid structure, a process that can be best described as molecular mimicry. What happens next is the immune system forms antibodies against both the coronavirus and ACTH, and the antibody-bound ACTH becomes completely ineffective at stimulating the release of cortisol, resulting in hypocortisolism [3]. It is postulated that the virulence of SARS-CoV-2 may be related to the degree of antigenic similarity between ACTH and its molecular mimic within the virus [2]. A condition referred to as critical illness-related corticosteroid insufficiency (CIRCI) occurs in patients with an acute stress response, leading to reduced cortisol levels that do not match the severity of the disease. CIRCI is presumed to occur in several critical conditions, including sepsis and septic shock, severe community-acquired pneumonia, acute respiratory distress syndrome, cardiac arrest, head injury, trauma, burns, and following major surgery [4]. Because of the mechanisms involved in suppressing the release of cortisol, Siejka recommends early use of steroids not only to replace what is deficient, but also because hydrocortisone protects against endothelial barrier dysfunction, which is strongly associated with COVID-19 [1]. Early Treatment of COVID-19 with Steroids to Inhibit Disease Progression Early in the COVID-19 crisis, it was the general consensus that steroids given too early in the disease process might suppress the immune system’s ability to fight the infection and reduce viral replication. As noted above, antibodies are produced against ACTH during SARS-CoV-2 infection. These antibodies interfere with ACTH’s normal signaling to trigger cortisol production as part of the stress response. Lower cortisol levels then provide feedback to the hypothalamus and pituitary to increase the production of ACTH to release more cortisol. Instead, increased ACTH production increases ACTH antibodies that do nothing to fight the viral infection. It is proposed by both Siejka and Wheatland that if corticosteroids are administered early, shortly after the start of the infection, ACTH production and therefore antibody production toward ACTH would decrease, freeing up the immune system’s antibody response to fight against the virus rather than ACTH [1, 2]. The increase in exogenously-administered corticosteroids may also help fine-tune the inflammatory response early, which would keep tissue damage under control. Early intervention with lower potency steroids like hydrocortisone, may act as a replacement for what is missing due to the interference of cortisol production by the virus. Perhaps this proves the adage that, “an ounce of prevention is worth a pound of cure.” Studies Related to SARS and Adrenal Insufficiency One small study on 28 hospital patients demonstrated that adrenal response to infection with SARS-CoV-2 was unexpectedly decreased – patients had low plasma cortisol and ACTH levels consistent with central adrenal insufficiency. Lower plasma cortisol and ACTH levels were consistent with higher disease severity, suggesting a direct link between COVID-19 and impaired glucocorticoid response [3]. Interestingly, ACE2 receptors, the gateway for SARS-CoV-2 entry into cells, are expressed in the hypothalamus, pituitary and adrenal glands, allowing the virus to enter these tissues. Autopsy studies on patients who died from the SARS-CoV-1 infection showed evidence of the viral genome in hypothalamic tissue. In a recent case study, a 51-year-old male presented to the emergency department complaining of two episodes of vomiting. He had received a positive COVID-19 test via polymerase chain reaction testing 10 days prior but was asymptomatic with stable vital signs and a normal chest X-ray at the time of diagnosis. No laboratory studies were done, and he was instructed to quarantine at home. When he presented to the ER, he was hypotensive with multiple electrolyte derangements that could not be substantiated by only two episodes of vomiting. Adrenal insufficiency was confirmed by low morning cortisol levels and an ACTH stimulation test. The patient was started on 20 mg of prednisolone daily with subsequent improvement in blood pressure and sodium levels [5]. In another case study, a 47-year-old male with a recent diagnosis of COVID-19, developed new onset central hypocortisolism in the convalescent phase of a mild case of COVID-19. He was sent to an isolation facility but returned to the hospital one week later due to a new onset seizure. The patient’s vital signs were normal, and he was well-oxygenated on room air. His bloodwork revealed elevated eosinophils and he complained of new onset persistent dyspepsia. Hypocortisolism was suspected and confirmed with a serum cortisol of 19 (normal range = 133-537 nmol/L) and an ACTH of 7.1 (normal range = 10.0-60.0 ng/L). He also presented with elevated thyroxine and thyroid stimulating hormone (TSH). This led his physicians to believe that the hypocortisolism was due to effects within the hypothalamic-pituitary pathways, affecting both adrenal and thyroid function [6]. SARS-Induced Endocrinopathy The deleterious impact of SARS-CoV-2 on the various organs within the endocrine system are becoming clearer as further research reveals the far-reaching health consequences of the virus. As we develop an understanding of the extent of these effects, we can better intervene with appropriate therapy. We understand from current research that SARS-CoV-2 can induce new onset or worsen existing conditions, such as diabetes mellitus, trigger hypocortisolism, and contribute to thyroid and reproductive aberrations [7]. The available data suggest that effects on endocrine tissues can occur either from direct viral damage or from immune-mediated mechanisms on endocrine tissues precipitated by the SARS-CoV-2 virus [7]. Diabetes mellitus increases the risk for the development of COVID-19 complications and adverse outcomes. In reviewing the effects of SARS-CoV-1, it was suggested that the virus could directly damage pancreatic cells that express ACE2 receptors, leading to a state of hyperglycemia, which reduces immune response and increases organ damage and systemic complications [8]. In a recent article in Endocrine, Mongioi et al hypothesized that patients with COVID-19 may be subject to virus-mediated pancreatic damage, resulting in the development of diabetes. It is uncertain at this point if the onset of diabetes is permanent or a transient effect of the virus that will eventually resolve with recovery from the infection [8]. Little is known about the effects of SARS-CoV-2 on thyroid function; however, some research has revealed a relationship between SARS-CoV-1 and central hypothyroidism secondary to hypothalamic-pituitary dysfunction resulting in low TSH. In secondary hypothyroidism, if the thyroid is not receiving the message from TSH to stimulate the production of thyroid hormones, T3 and T4 will also be low. SARS-CoV-1 did not directly invade the thyroid; however, tissue studies revealed injury to the follicular epithelium with an increase in cell apoptosis, suggesting that the damage was likely mediated by an immune response against the thyroid [8]. Direct damage to thyroid tissue could result in impaired function and a decrease in thyroid hormones and calcitonin levels as compared to controls. It appears that both primary and secondary hypothyroidism may be caused by the virus. The effects of COVID-19 on thyroid function appear to be transient and resolve shortly after recovery from the viral infection [7]. Though no evidence exists that SARS-CoV-2 infects the ovaries, increases in serum prolactin, follicle-stimulating hormone, and luteinizing hormone with a reduction in estradiol and progesterone levels was noted in SARS patients as compared to controls. We do know that ACE2 is highly expressed in testicular tissue and past studies have found tissue damage indicative of orchitis on autopsy of six patients who died with SARS-CoV-1. Tissue effects may also be representative of immune-mediated damage triggered by the presence of the virus [8]. Some researchers suspect that testicular tissue provides an additional site of infection and may increase viral load in males as compared to females. The hypothalamic and pituitary tissue also have ACE2 receptors and can be sites of SARS-CoV-2 infection if the virus enters the brain. As previously mentioned, it is suspected that SARS-CoV-2 can enter the brain via the olfactory pathway where it gains access to the brain across the porous cribriform plate. The virus can then enter the hypothalamus fairly easily as the blood-brain barrier is permeable near this structure. Infection of the hypothalamus may result in hypophysitis (inflammation of the hypothalamus) and would have broad effects across the entire endocrine system. Long COVID and Adrenal Insufficiency Adrenal insufficiency tends to develop in COVID-19 patients during the late stage of the disease and appears to be secondary to hypophysitis or direct damage to the hypothalamus from the SARS-CoV-2 virus. In 2005, Leow et al explored the function of the HPA axis in 61 SARS-CoV-1 survivors by evaluating serum electrolytes, cortisol, ACTH levels, and 24-hour urinary-free cortisol three months after the acute infection. Adrenal insufficiency was defined as cortisol values <550 nmol/L 30 minutes after an ACTH stimulation test. Nearly 40% of patients tested had hypocortisolism and among them 83% had central adrenal insufficiency, which is secondary to ACTH deficiency [9]. Of those with hypocortisolism, 62.5% recovered within one year paralleled by the resolution of orthostatic hypotension and an overall improved sense of well-being. The majority of the patients in the study exhibited cortisol dynamics analogous to patterns seen in chronic fatigue syndrome, post-traumatic stress disorder, and fibromyalgia [9]. It is important to note that patients may also experience hypocortisolism due to treatment with dexamethasone to reduce inflammation during active infection. Treatment of COVID-19 with potent steroids to address the immunoinflammatory response to the infection may result in short-term adrenal insufficiency that may require evaluation and supportive care. In the 2005 study by Leow et al referenced above, the majority of the patients studied had not been treated with steroids during the course of their illness. Four of the six who had received high-dose parenteral glucocorticoids did not develop post-SARS hypocortisolism as demonstrated by the lack of prolonged suppression of the HPA axis as revealed in serial ACTH stimulation tests [9]. Addressing Long COVID through HPA Axis Support Many of the symptoms of post-viral syndromes interfere with our ability to thrive, which indicates involvement of the autonomic nervous system (ANS), leaving us with orthostatic intolerance, cognitive dysfunction, muscle weakness, fatigue, dizziness, and heart rate abnormalities. Although isolating where some of these symptoms may be coming from is a starting point to treatment, it is necessary to consider the contribution of other organ systems to each dysfunction. Many of the symptoms associated with autonomic dysfunction may be related to the ability of the mitochondria to make energy and support metabolic processes. Involvement of the HPA axis with the sympathetic branch of the ANS contributes to maintaining adequate blood sugar and blood pressure, as well as inhibiting inflammation and supporting a balanced immune system response. An appropriate response of the HPA axis during an acute stressor is necessary for survival, but frequent and prolonged activation can alter the functional tone of the stress response reducing the ability of the HPA axis to respond efficiently and effectively [10]. Post-viral illness is difficult to address in that it cannot be cured by treating only one symptom or one system. Genetic susceptibility, nutritional status, and state of health prior to infection will likely play a role in the development of long COVID, and the supportive care to pull through the long-term sequelae of this virus will require a multi-pronged approach. Our ability to respond to stressors involves the sympathoadrenal system and the HPA axis, and interactions between these two systems play a central role in adaptation. Healthy function of the HPA axis is dependent on healthy mitochondria for steroid hormone conversion and an intact ANS to support a normal stress response. Long-term changes to the HPA axis may occur in response to the stress of an acute illness that has altered the metabolism of cortisol, leading to a reduced capacity to respond to stress [4]. To address the oncoming tidal wave of COVID-19 survivors who have ongoing symptoms, supporting the HPA axis through nutrition, supplementation, adaptogenic herbs, glandulars, and hydrocortisone if needed, will likely offer some relief. Options for support of the nervous system, immune system, and mitochondria have been outlined in parts one through three of this series. Testing the HPA Axis Aside from infection with the SARS-CoV-2 virus, the stress of the pandemic itself has been extensive. Fear of infection, lockdowns, social isolation, financial instability, and emotional distress have taken a toll on our collective psyche. The extraordinary amount of stress that we have been under can certainly have lasting consequences in chronic activation of the stress response systems, leading to ongoing dysfunction of the HPA axis. For those who have been infected with the virus, early testing of the HPA axis offers another opportunity for intervention and recovery from COVID-19 and can be easily done at home through a multi-point salivary test measuring cortisol output throughout the day. As the above-referenced studies indicate, developing hypocortisolism is a real possibility after the infection with SARS-CoV-2 and may likely be a major contributor to the symptoms of long COVID. Testing for and addressing this issue is key to a full recovery. In addition to multi-point cortisol measurements, our clinic can also provide testing to assess melatonin levels, dehydroepiandrosterone sulfate (DHEA-S), high-sensitivity C-reactive protein (hsCRP), vitamin D, sex hormones, thyroid hormones, and neurotransmitters. Melatonin increases in response to darkness but may be compromised due to dysregulation within the circadian rhythm. In addition to regulating sleep, melatonin is a potent antioxidant that can help to neutralize reactive oxygen species produced during inflammation. DHEA-S functions in the brain and nervous system as a neurosteroid, is a potent immune-modulating hormone and functions as a counter-regulatory hormone to cortisol. The main neurobiological effects of DHEA-S in the brain include neuroprotection, neurogenesis, apoptosis, catecholamine synthesis and secretion, and antioxidant and anti-inflammatory effects. Measuring sex hormones and thyroid markers can provide much needed data that may help to address the symptoms associated with post-viral illness. While an initial infection can resolve, it can leave behind an inflammatory footprint that propagates further damage. Measuring hsCRP can provide us with information regarding general inflammation and infection. hsCRP can be readily measured in a dried blood spot (DBS) sample alongside other cardiovascular and metabolic markers. Healthy vitamin D levels are associated with a robust and balanced immune response and can also be measured in DBS. Our clinic can also provide testing to assess neurotransmitters that impact mood, cognitive ability, and sleep. Neurotransmitters are responsible for functionally integrating the immune and endocrine systems, indicating that neurotransmitter imbalances often reach beyond the brain. As we face the burgeoning issue of long COVID, the approach to treatment will involve addressing inflammation and dysregulation within the central nervous system, autoimmune issues, mitochondrial function, and hormone and HPA axis dysregulation. We have a long road ahead of us and there will likely be many who suffer from the long-term consequences of this pandemic – those who acquired the infection and those who simply lived through it. Our personal timelines may be forever referred to by pre-COVID and post-COVID events. My hope is that through the efforts of the many brilliant minds in medical research and through the practice of preventive and interventional therapies, we have learned how to better prepare for and respond to a novel virus. Early treatment for viral infections and immune system support are still important as coming up with new vaccines for each novel virus may not be practical, timely or safe. We may not be able to prevent infection, but by investing in our health every day, we may effectively support our recovery. References 1. Siejka A, Barabutis N. Adrenal insufficiency in the COVID-19 era. Am J Physiol Endocrinol Metab. 2021;320(4):E784-E785. 2. Wheatland R. Molecular mimicry of ACTH in SARS – implications for corticosteroid treatment and prophylaxis. Med Hypotheses. 2004;63(5):855-862. 3. Alzahrani AS, Mukhtar N, Aljomaiah A, et al. The impact of COVID-19 viral infection on the hypothalamic-pituitary-adrenal axis. Endocr Pract. 2021;27(2):83-89. 4. Annane D, Pastores SM, Arlt W, et al. Critical illness-related corticosteroid insufficiency (CIRCI): a narrative review from a multispecialty task force of the Society of Critical Care Medicine (SCCM) and the European Society of Intensive Care Medicine (ESICM). Intensive Care Med. 2017;43(12):1781-1792. 5. Hashim M, Athar A, Gaba WH. New onset adrenal insufficiency in a patient with COVID-19. BMJ Case Rep. 2021;14(1):e237690. 6. Chua MWJ, Chua MPW. Delayed onset of central hypocortisolism in a patient recovering from COVID-19. AACE Clin Case Rep. 2021;7(1):2-5. 7. Kothandaraman N, Rengaraj A, Xue B, et al. COVID-19 endocrinopathy with hindsight from SARS. Am J Physiol Endocrinol Metab. 2021;320(1):E139-E150. 8. Mongioì LM, Barbagallo F, Condorelli RA, et al. Possible long-term endocrine-metabolic complications in COVID-19: lesson from the SARS model. Endocrine. 2020;68(3):467-470. 9. Leow MK‐S, Kwek DS-K, Ng AW-K, et al. Hypocortisolism in survivors of severe acute respiratory syndrome (SARS). Clin Endocrinol (Oxf). 2005;63(2):197-202. 10. Steenblock C, Todorov V, Kanczkowski W, et al. Severe acute respiratory syndrome coronavirus 2 (SARS-CoV-2) and the neuroendocrine stress axis. Mol Psychiatry. 2020;25(8):1611-1617.
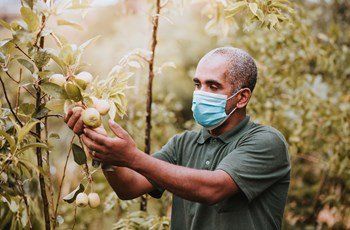
In parts one and two of this series, we looked at the issues related to long COVID and its impact on the nervous and the immune systems. The effects of COVID-19 on the nervous system can present as localized effects such as loss of smell and taste to chronic fatigue, headaches, postural orthostatic tachycardia syndrome, and cognitive issues. The potential to trigger an autoimmune reaction is a very real possibility with any infection and is stimulated by molecular mimicry, bystander activation, and viral persistence. The presence of a healthy and diverse gut and lung microbiome helps to regulate the immune system and supports a robust and balanced innate immune response. The most common reported symptom of long COVID is fatigue and the overall symptom picture resembles that of myalgic encephalomyelitis/chronic fatigue syndrome (ME/CFS), which is often triggered by a viral infection. Fatigue can be described as lack of energy; and when we think of energy production, we think of the mitochondria. In addition to being the powerhouses of the cell, mitochondria are also involved in maintaining cell immunity, homeostasis, cell survival, and cell death. Mitochondria also play a role in cell apoptosis (programmed cell death), calcium signaling, regulation of cellular membrane potential, and steroid synthesis [1]. SARS-CoV-2 hijacks the mitochondria and uses it for protection and viral replication, diverting resources from supporting normal cellular function to now serving as a viral factory. Decreased mitochondrial function means less cellular energy, resulting in generalized fatigue, muscle weakness, and brain fog on a broader scale. In part three of this series, we will explore the effect of viral infection on mitochondrial function, how SARS-CoV-2 specifically affects mitochondria, resulting in symptoms of long COVID and what we can do to support mitochondrial function to prevent the long-term effects of COVID-19. Basic Overview of Mitochondrial Function Mitochondria are responsible for the majority of energy production in the form of adenosine triphosphate (ATP), which is readily used by cells as a source of chemical energy. Cellular respiration is the process by which mitochondria produce chemical energy from glucose. Through the process of glycolysis, one molecule of glucose is converted to two molecules of pyruvate, two molecules of nicotinamide adenine dinucleotide (NADH) and two molecules of ATP. The pyruvate enters the mitochondria where it is converted into acetyl-coenzyme-A, which enters the tricarboxylic acid (TCA or Krebs) cycle and produces two additional molecules of ATP. The energy-rich products of the TCA cycle, NADH and flavin adenine dinucleotide, undergo oxidative phosphorylation (OXPHOS) via the electron transport chain (ETC), producing water and 32 additional molecules of ATP. The entire process of cellular respiration results in approximately 36 molecules of ATP to support cellular energy needs [2]. The process of glycolysis, which is the first step in cellular respiration, occurs in the cytosol of the cell. If there is enough oxygen available for cellular respiration, the process of energy production will advance to the TCA cycle and OXPHOS along the ETC. However, if oxygen is low, glycolysis results in the production of two molecules of ATP and lactic acid. Anaerobic glycolysis can occur for quick energy production during extreme exercise or illness where oxygen delivery to cells is deficient. The reliance on anaerobic glycolysis as a means of energy production can result in fatigue and muscle pain due to the buildup of lactic acid [2]. Another form of energy production is through aerobic glycolysis also known as the Warburg effect. Once thought to occur solely in cancer cells, aerobic glycolysis may occur in specific immune cells in response to signaling events and the physiological environment. In the field of immunometabolism, it has been observed that metabolic shifts in immune cells may occur to facilitate specific immune responses, and many viruses induce metabolic reprogramming in host cells similar to the Warburg effect observed in cancer cells to produce a quick and readily available source of energy [3, 4]. Viral Influence on Mitochondrial Function Under the influence of a viral infection, mitochondria experience a loss of integrity in structure and function and can trigger an immune response that activates the production of inflammasomes, which may contribute to autoimmunity and ongoing inflammatory reactions [5]. Inflammasomes are intracellular proteins that trigger the activation of inflammatory cytokines. Mitochondria play a central role in the host response to viral infection and immunity, and function as a platform for immune signaling by engaging the interferon system, which operates as a liaison between the innate and adaptive immune systems [6]. SARS-CoV-2 has the ability to disable the initial immune response by inhibiting the production of interferons. Reduced interferon production delays the release of natural killer cells and stagnates T cell response. Hepatitis C, hepatitis B, adenoviruses, herpes simplex virus-I, Epstein Barr virus, cytomegalovirus, influenza A and coronaviruses have adaptations that allow them to inhibit the interferon pathway to prolong survival and evade the immune system, leaving them with a greater chance to replicate and spread [6]. Viral influence on mitochondrial activities can lead to altered energy levels by changing mitochondrial density and function, resulting in suboptimal energy output [7]. Mitochondria also produce reactive oxygen species (ROS) as a by-product of the process of oxidative phosphorylation. Once thought to have only harmful effects, it is now known that ROS function as signaling molecules, which increase the production of antioxidants, promote apoptosis, and trigger the production of new mitochondria. Mitochondrial ROS also induce mitochondrial anti-viral signaling (MAVS), resulting in induction of type I interferons, heralded as key regulators of antiviral activity [5]. ROS are a normal product of oxidative phosphorylation and can be neutralized through adequate availability of antioxidants; however, this process can become overwhelmed during infection, when ROS production increases beyond the body’s ability to control the oxidative stress. Infection can also interfere with the energy-producing pathway of the mitochondria, leaving cells and tissues with an energy deficit and an excess of ROS. S ARS-CoV-2 Hijacks Mitochondrial Function Host response against viral infections depends on optimal mitochondrial function but the presence of SARS-CoV-2 can cause structural and metabolic changes within the mitochondria, interfering with an appropriate immune response. Host cell metabolism and activation of signaling pathways is reprogrammed by SARS-CoV-2 viral proteins [4]. SARS-CoV-2 uses its spike glycoprotein, assisted by host transmembrane serine protease 2 (TMPRSS2), to gain access to cells via the angiotensin-converting enzyme-2 (ACE2) receptor on the host cell. Binding of this receptor by the virus decreases the production of ACE2, which has a regulatory effect on mitochondrial function. Less ACE2 results in less ATP production [6]. Once the virus has entered the cell, viral RNA, RNA transcriptase, and viral open-reading frames (ORFs) enter the mitochondria to hijack and manipulate function. ORFs are segments of DNA or RNA from the virus that code for particular proteins. Viral ORFs interact with mitochondrial proteins to directly manipulate mitochondrial function to evade host cell immunity, suppress immune response, and support viral replication [6]. The presence of the virus also creates stress within the mitochondria, leading to the formation of double-walled vesicles, which give the virus a place to hide and replicate. These vesicles function like portable organelles, taking viral information to the endoplasmic reticulum to further aid in viral replication [6]. SARS-CoV-2 also promotes a metabolic shift to aerobic glycolysis to facilitate viral replication and survival. The Warburg effect appears to be involved in several processes during COVID-19 infection [4]. In response to hypoxia, the Warburg effect is induced in lung endothelial cells, which in the presence of atherosclerosis, can lead to vasoconstriction and thrombosis. Initially, aerobic glycolysis supports the activation of pro-inflammatory pathways that are needed to mobilize defenses against infections; however, a subsequent shift to the OXPHOS pathway is also needed to promote anti-inflammatory pathways that are reparative. Aging, cardiovascular disease, metabolic syndrome, type II diabetes, obesity, hypertension, and chronic kidney disease along with mitochondrial senescence contribute to a state of chronic inflammation, which promotes the Warburg effect and prevents the metabolic transition to OXPHOS to decrease inflammation and initiate repair mechanisms [4]. Aging, Comorbidities, and Mitochondrial Function Factors that favorably contribute to the takeover of mitochondrial function by the SARS-CoV-2 virus are aging, chronic inflammation, and genetic susceptibility. The aging process results in less mitochondria and lower production of ATP with a decrease in autophagy of mitochondria, which contributes to unregulated inflammasome activity leading to chronic inflammation. The process of aging is marked by the progressive decline in cellular function, which increases susceptibility to age-related morbidity and mortality [6]. One of the hallmarks of aging is mitochondrial dysfunction, which induces senescence and contributes to the process of inflammaging. Senescence occurs when cells lose the power to divide and grow, and inflammaging is the increase in systemic inflammation as one ages. The overall effect reduces mitochondrial capacity by about 50%, resulting in fatigue and muscle weakness [6]. Many of the conditions that are considered comorbidities for SARS-CoV-2 contribute to a state of chronic inflammation. Diabetes, heart disease, obesity, and metabolic diseases are commonly present with mitochondrial dysfunction. Mitochondrial genetic mutations may also be a contributing factor in determining the severity of post-viral sequelae related to mitochondrial function. While there are no studies on the long-term effects of SARS-COV-2 on mitochondrial function, past studies on ME/CFS strongly implicate mitochondrial dysfunction as a central cause of ME/CFS symptoms [8, 9]. A renewed interest in the causes of post-viral syndromes will likely be forthcoming as we progress through this pandemic. Mitochondrial Issues in Post-Viral Syndromes and ME/CFS Although no single cause has been associated with ME/CFS, viral infections have often been cited to trigger the onset of this disorder. In the case of SARS-CoV-2 and other viruses, there may be a direct impact on mitochondrial function from the virus itself [10]. The oxidative stress that occurs during an infection can leave mitochondria in a state of dysfunction. The mitochondria of our cells are responsible for the production of ATP and produce 90-95% of the body’s total energy [11]. ATP drives all the necessary chemical reactions in the body and if there is a shortage of ATP production, basic biochemical reactions may not be optimized. As a point of comparison, mitochondrial diseases associated with genetic mutations manifest as fatigue, muscle weakness, and cognitive decline along with waxing and waning energy patterns typical of ME/CFS. While there are no definitive markers to identify ME/CFS specifically, there are a range of different markers that can be used to assess mitochondrial function including mitochondrial proteins, production of ATP, and oxygen consumption of live plated cells. The earliest evidence of the relationship between ME/CFS and mitochondrial issues was seen in structural changes of skeletal muscle cell mitochondria in peripheral blood mononuclear cells. Also noted was the decrease in OXPHOS and an increase in aerobic glycolysis, resulting in less ATP production in ME/CFS patients as compared to controls. As reported by the Journal of Translational Medicine, Sweetman et al concluded that mitochondrial dysfunction can happen via oxidative damage as might occur during an extreme inflammatory reaction to an infection [12]. In a 2019 article in Mitochondrion, Robert Naviaux, MD, PhD, discusses the cell danger response (CDR) and its connection to environmental health, mitochondrial function, and chronic illness. The CDR is a universal response to environmental threat, stress, infection or injury, and it is the mitochondria that sense and respond to changes in the cellular environment and mediate the regulators of the CDR that signal safety or danger within the cell. Once initiated, the CDR cannot be turned off and must cycle through to completion to effectively resolve the danger response. If the CDR persists abnormally, whole body metabolism, the gut microbiome, and multiple organ systems become impaired and chronic disease can emerge. Previous illness, stress, environmental toxins, chronic inflammatory illnesses, hormonal imbalances, autoimmune disorders, and allergies can impede the process of resolution of the CDR [13]. As much of the CDR is mediated by the mitochondria, supporting mitochondrial function, and employing general habits of good health can support a healthy CDR and potentially resolve chronic illness. Mitochondrial Support To offset the production of ROS within the mitochondria, enzymes, and coenzymes such as vitamin E and CoQ10 (ubiquinone) help to remove ROS to prevent damage. If essential nutrients, such as vitamin E and CoQ10 are deficient, removal of the ROS is impaired and damage through oxidative stress occurs. CoQ10 is the only lipid-soluble antioxidant that is produced endogenously in humans [11]. Ubiquinol is the reduced form of CoQ10 and acts as an antioxidant by reducing ROS and regenerating other antioxidants. It is also found in the highest concentration in the most metabolically active tissues such as the heart, liver, and muscle. Deficiencies of CoQ10 can occur with less endogenous production as we age and can be depleted with certain medications like statins to reduce cholesterol. CoQ10 deficiency may also be associated with dysfunctional OXPHOS, which can occur in ME/CFS. A study of 58 ME/CFS participants and 22 healthy controls, showed that the ME/CFS participants had lower overall levels of CoQ10, and their degree of fatigue was associated with a lower concentration of CoQ10 in plasma. It was also noted that ATP levels were significantly decreased while lipid peroxidation was increased, indicating mitochondrial dysfunction [11]. In a study out of Spain, Castro-Marrero et al evaluated the effects of CoQ10 and reduced NADH supplementation on the symptoms associated with ME/CFS. Seventy-three female participants were randomized to either the CoQ10+NADH or placebo group. In addition to improvements in fatigue according to the Fatigue Impact Scale, after eight weeks supplementing with 200 mg of CoQ10 and 20 mg of NADH, participants in the supplement group showed a decrease in oxidative damage, improvement in mitochondrial function, and enhanced energy [14]. CoQ10 and NADH can stimulate energy production by replenishing depleted cellular stores of ATP, and together they can act as free radical scavengers that can reduce lipid peroxidation and DNA damage caused by oxidative stress. Mitochondria are very susceptible to environmental toxins, nutrient deficiencies, and oxidative stress [15]. Additional support for mitochondrial function includes acetyl-L-carnitine, pyrroloquinoline quinone, vitamin C, choline, α-lipoic acid, α-ketoglutaric acid, resveratrol, N-acetyl cysteine, magnesium, and a quality multivitamin and mineral complex. Several professional product lines have mitochondrial support products that include most of the above-listed nutrients. In Summary Age and health status prior to infection can be a predictor of outcomes and may help us to determine who will develop long COVID. Metabolic issues associated with aging, obesity, and a sedentary lifestyle are not supportive of mitochondrial health. Viruses can manipulate the energy-producing pathway of the mitochondria to favor glycolysis over OXPHOS. If the mitochondria are already in a state of dysfunction due to preexisting health issues and oxidative stress from low-level inflammation, their ability to keep up with energy demands becomes severely compromised [10]. The hijacking of the mitochondria to serve the needs of the virus structurally and functionally changes the mitochondria, resulting in ongoing inflammation and a reduced ability to support the energy needs of cells. Symptoms such as fatigue and weakness, are often the result of these cellular changes. COVID-19 long-haulers may experience excessive oxidative damage in response to extreme inflammation generated by the infection; however, poor mitochondrial status and deficiencies in nutrients that are needed to quench ROS may also be prevalent among those who experienced only mild to moderate illness. This might explain why the severity of COVID-19 does not seem to predict who experiences post-viral syndrome or long COVID. Having less metabolic reserve due to stress, inadequate sleep, hypothalamic-pituitary-adrenal (HPA) axis dysfunction, poor diet, chronic inflammation, a constant low level of oxidative stress, and the existence of comorbidities may lead to the development of long COVID even if the acute infection is mild to moderate. References 1. Rogers K. Mitochondrion. Definition, function, structure & facts. Encyclopedia Britannica. https://www.britannica.com/science/mitochondrion. Accessed April 30, 2021. 2. BD Editors. Mitochondria. Biology Dictionary. https://biologydictionary.net/mitochondria/. Accessed April 30, 2021. 3. Jones W, Bianchi K. Aerobic glycolysis: beyond proliferation. Front Immunol. 2015;6:227. 4. Icard P, Lincet H, Wu Z, et al. The key role of Warburg effect in SARS-CoV-2 replication and associated inflammatory response. Biochimie. 2021;180:169-177. 5. Iwasaki Y, Takeshima Y, Fujio K. Basic mechanism of immune system activation by mitochondria. Immunol Med. 2020;43(4):142-147. 6. Singh KK, Chaubey G, Chen JY, et al. Decoding SARS-CoV-2 hijacking of host mitochondria in COVID-19 pathogenesis. Am J Physiol Cell Physiol. 2020;319(2):C258-C267. 7. Ganji R, Reddy PH. Impact of COVID-19 on mitochondrial-based immunity in aging and age-related diseases. Front Aging Neurosci. 2021;12:614650. 8. Booth NE, Myhill S, McLaren-Howard J. Mitochondrial dysfunction and the pathophysiology of myalgic encephalomyelitis/chronic fatigue syndrome (ME/CFS). Int J Clin Exp Med. 2012;5(3):208-220. 9. Myhill S, Booth NE, McLaren-Howard J. Chronic fatigue syndrome and mitochondrial dysfunction. Int J Clin Exp Med. 2009;2(1):1-16. 10. Nunn AVW, Guy GW, Brysch W, et al. SARS-CoV-2 and mitochondrial health: implications of lifestyle and ageing. Immune Ageing. 2020;17(1):3 3. 11. Wood E, Hall KH, Tate W. Role of mitochondria, oxidative stress and the response to antioxidants in myalgic encephalomyelitis/chronic fatigue syndrome: a possible approach to SARS-CoV-2 ‘long-haulers’? Chronic Dis Transl Med. 2021;17(1):14-26. 12. Sweetman E, Kleffmann T, Edgar C, et al. A SWATH-MS analysis of myalgic encephalomyelitis/chronic fatigue syndrome peripheral blood mononuclear cell proteomes reveals mitochondrial dysfunction. J Transl Med. 2020;18(1):365. 13. Naviaux RK. Perspective: cell danger response biology—the new science that connects environmental health with mitochondria and the rising tide of chronic illness. Mitochondrion. 2020;51:40–45. 14. Castro-Marrero J, Cordero MD, Segundo MJ, et al. Does oral coenzyme Q10 plus NADH supplementation improve fatigue and biochemical parameters in chronic fatigue syndrome? Antioxid Redox Signal. 2015;22(8):679-685. 15. Pizzorno J. Mitochondria—fundamental to life and health. Integr Med (Encinitas). 2014;13(2 ):8-15.
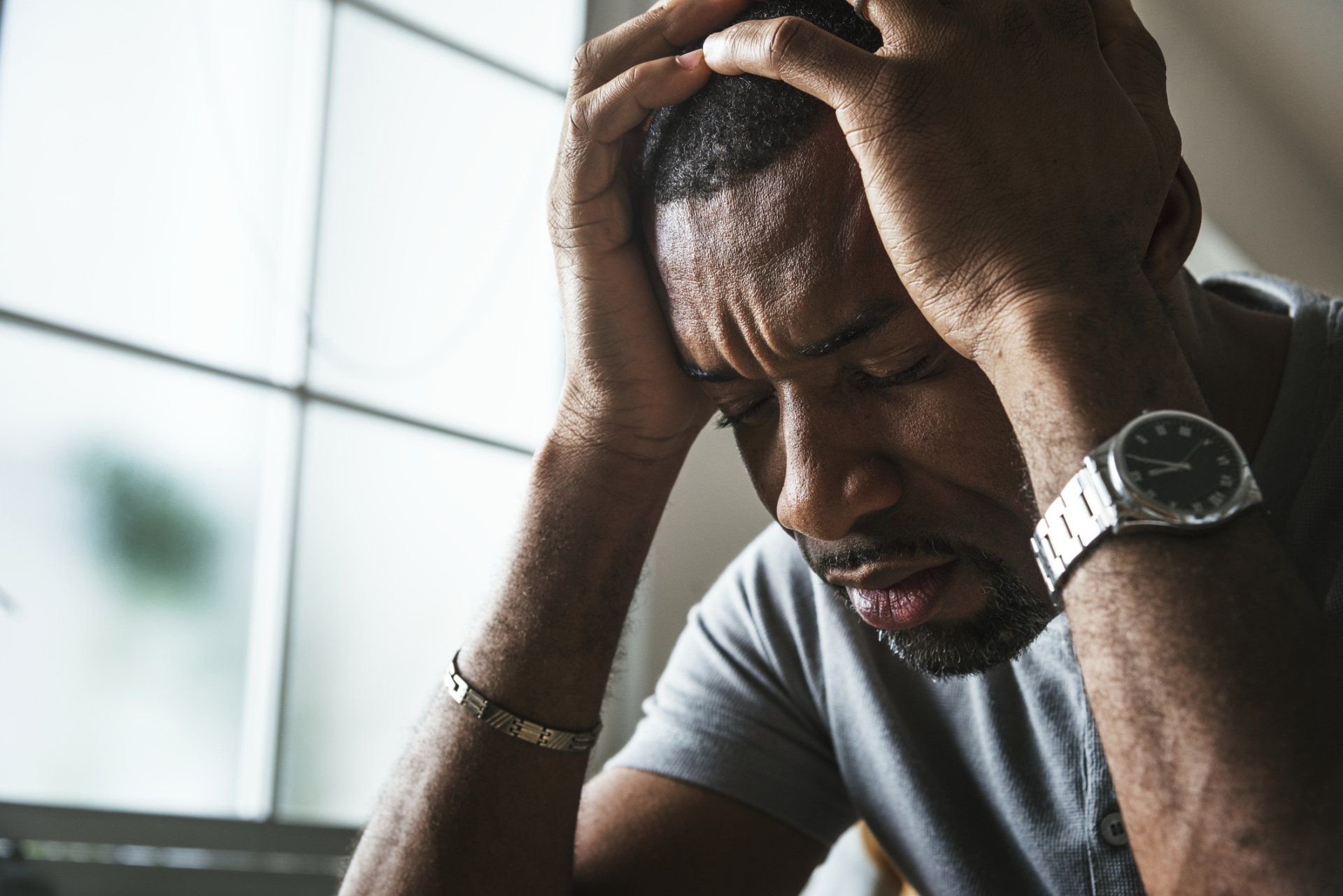
In the first part of this series on post-COVID illness, I reviewed some of the issues of post-viral syndromes and their relationship to the current pandemic with a deeper look into the effects of SARS-CoV-2 on the nervous system. Many of the symptoms associated with long COVID present as myalgic encephalomyelitis/chronic fatigue syndrome (ME/CFS) with some additional symptoms specifically associated with COVID-19. Post-viral syndromes are not new, but it is not completely clear as to why some patients experience lasting symptoms after a viral infection and some do not. We do know that immune system activation by a virus increases systemic inflammation, oxidative stress, and tissue damage. We also know that our genetics play a role, as evidenced by the tendency to develop autoimmune conditions for those with specific human leukocyte antigen (HLA) phenotypes that can be triggered by certain infections. The tendency to develop ongoing inflammation and lingering symptoms after an infection might be relative to baseline inflammation and immune system balance upon encountering the virus. The potential to unmask underlying chronic infections may also contribute to the symptoms of long COVID. We are all exposed to viruses and other infectious agents throughout our lifetime that can exist in a latent state only to reactivate during a time of stress and immune dysregulation, which adds to the complexity of post-viral syndromes including long COVID. SARS-CoV-2 and the Immune System Viruses have evolved to evade the immune system in many ways. SARS-CoV-2 disables the innate immune system during early infection by blocking the ability of Toll-like receptors (TLR) to signal the production of interferon-I (IFN-I), which is crucial for reducing viral replication and spread [1,2,3]. This gives the virus a greater chance to replicate during early infection, leading to higher viral titers and the potential for a heightened inflammatory reaction later. Innate immune response during early infection determines what will happen later in the disease process and possibly beyond recovery from the acute infection. Reducing viral load early may decrease the chance of continued inflammation, autoimmunity, and poor viral clearance. In a mouse model of SARS-CoV-2 infection, local IFN responses in the lungs were delayed relative to peak viral replication, which greatly reduced viral clearance and was associated with the development of cytokine release syndrome (CRS) [1]. Through various mechanisms, coronaviruses are known to inhibit the early production of IFN but increase the production of nuclear factor kappa light chain enhancer of activated B cells (NF-KB), resulting in production of inflammatory cytokines and chemokines. IFN acts as a central liaison between the innate and adaptive immune systems and signaling can trigger various messages that dictate how and when the innate and adaptive immune systems respond to viral infection. Use of INF during early infection has shown some promise to reduce viral replication; however, the timing of use is critical. Introducing INF too late in the disease process may result in a heightened inflammatory response that may contribute to the development of a cytokine storm and interfere with effective recovery [1]. Given the safety concerns regarding the use of IFN as a treatment option for COVID-19, considering nutraceuticals that can boost IFN-I might be a better option. McCarty et al identify key mechanisms of action of ferulic or lipoic acid, spirulina, N-acetyl cysteine (NAC), selenium, glucosamine, zinc, beta-glucan, and elderberry, which can interfere with the ability of the virus to inhibit IFN-I through support of TLR activity and boosting of IFN-I production [2]. We are familiar with most of these nutraceuticals as immune system support and specific dosages are highlighted in the article. Recent studies out of Casanova’s lab at The Rockefeller University have also discovered the relationship between genetic mutations and inborn errors of IFN-I immunity and the severity of COVID-19. They have also noted some patients with severe COVID-19 can develop autoantibodies to IFN-I [4]. With a reduction in IFN-I activity whether through viral inhibition, genetic predisposition or the production of autoantibodies, the net effect is an increase in viral replication in the early phase of disease, which can have the effect of inducing an overly robust inflammatory reaction as the viral load increases. This overreaction may induce autoimmunity and/or chronic inflammation through bystander activation. Immune System Dysregulation/Autoimmunity There are three ways that a virus might induce autoimmunity: molecular mimicry, bystander activation, and viral persistence. Molecular mimicry between a virus or other pathogen and host proteins can lead to immunological cross-reactivity and subsequent attack on self. As stated in the Journal of Translational Autoimmunity, the existence of homology (molecular mimicry) between viral and human proteins is well-established in viral- or vaccine-induced autoimmunity [5]. As the body induces an immune response to the viral proteins, it also attacks similar proteins within body tissues that can extend beyond the initial infection, inciting ongoing inflammatory reactions in various tissues. The infection may have resolved; however, the inflammatory trigger is ongoing because the presence of the virus woke up the immune system in a way that initiated a reaction to proteins that were similar within the virus and within self. Much of this reaction is determined by the genetics, which is why people react differently to viruses and other pathogens. Some may recover without long-term sequelae while others battle ongoing symptoms after the acute infection. To highlight the potential issue of molecular mimicry and SARS-CoV-2, a recent article in Clinical Immunology by Aristo Vojdani, PhD, MSc, CLS, and Datis Kharrazian, PhD, DHSc, DC, MS, MMSc, FACN, examined cross-reactivity of viral antibodies with various body tissues. They tested five different blood specimens confirmed positive for SARS-CoV-2 immunoglobulin M (IgM) and immunoglobulin G (IgG) antibodies for anti-nuclear antibody (ANA), anti-extractable nuclear antigen (anti-ENA), anti-double stranded DNA, actin antibody, mitochondrial antibody, rheumatoid factor, and complement component 1q immune complexes. Three of the five specimens had elevated ANA, anti-ENA, actin, and mitochondrial antibodies. Further studies revealed 21 out of 50 tissue antigens reacted with the SARS-CoV-2 antibodies, indicating a cross-reaction between SARS-CoV-2 proteins and several tissue proteins. These tissues included the lungs, connective tissue, cardiovascular tissue, gastrointestinal (GI) tissue, and the nervous system [6]. This is a very timely representation of the potential for viruses to induce autoimmunity and is likely to have occurred in the past in relation to post-viral syndromes and chronic inflammation. Bystander activation can occur when virus-activated T cells kill infected cells along with uninfected neighboring cells. Cytokines and other inflammatory mediators that are released in the process increase tissue damage, which furthers the inflammatory reaction. Bystander activation occurs in the midst of an inflammatory milieu interacting with nearby tissues. This process can promote autoimmunity but may also result in a transient inflammatory event that eventually resolves [7,8]. This may explain why inflammation and autoimmunity might occur in tissues where there is no detection of the virus or other infectious agent. How and when the inflammation resolves may be dependent upon baseline inflammation and the ability of the immune system to self-regulate. The virus may also persist at a low level where it continues to be presented on host cells leading to prolonged immunopathology. Persistent viral infections can lead to immune-mediated tissue damage due to the constant presence of the viral antigen [8]. Viral persistence may also occur when the virus has learned to evade the immune system by decreasing the host cell’s ability to present surface antigen, which alerts the immune system and results in cell destruction. Another theory regarding viral persistence is T cell exhaustion and an increase in immune system regulator cells that increase immune tolerance, resulting in failure of the immune system to purge the virus [9]. Viruses may also develop a strategy to replicate without destroying the cell in which it occupies. Persistent viruses can also occupy differentiated or specialized cells creating specific dysfunction within those cells. Examples include viruses that interfere with the ability of neurons to make neurotransmitters, block endocrine cells from making hormones, and dysregulate the production and release of immune mediators that either suppress or enhance immune function [9]. This might explain the ongoing symptoms experienced by those with ME/CFS and other complex chronic illnesses. Viral persistence seems most concerning for long COVID as it leaves the virus to linger and develop more ways to evade the host’s immune response while interfering with homeostasis and cell function. The Gut-Lung Axis and Mucosal Immunity About 70% of our immune system resides in the GI tract. Our mucosal surfaces are where we encounter what is outside of ourselves. The mouth, sinuses, digestive tract, vaginal mucosa, and the lungs all directly interface with the outside world and it is through these surfaces that we encounter certain viruses. The gut microbiome directs immune function within the GI tract and beyond, and there is a bidirectional relationship between the GI tract and the lungs. The lungs also have their own microbiome that supports immune system function within the respiratory tract by modulating inflammation and creating homeostasis. The gut microbiome influences the lungs by way of the gut-lung axis and is linked through the mucosal immune system where gut bacteria-derived metabolites travel to the lungs via the mesenteric lymphatic system and general circulation. Likewise, conditions within the lungs can alter the microbiota in the GI tract [10]. Our greatest impact on mucosal immunity is through support of a healthy gut microbiome that promotes an effective immune response in the lungs via the gut-lung axis. In addition to a balanced immune response, the microbiome of various tissues supports immune tolerance so that we do not become excessively reactive to non-pathogenic antigens and regulates our response to infectious pathogens. The diversity and volume of bacteria that makes up the microbiome may influence the severity of COVID-19, as well as the magnitude of the immune system response to the infection. Imbalances in the makeup of the microbiome may also be implicated in persisting inflammatory symptoms associated with long COVID as indicated by a recent study comparing the gut flora of patients infected with and recovering from COVID-19. In this 100-patient cohort study, the bacterial composition of serial stool samples was compared to blood markers of inflammation. Of the 100 hospitalized patients, 27 were followed for up to 30 days past the clearance of the virus. Regardless of antimicrobial intervention, there were consistent differences in the microflora of COVID and non-COVID patients [11]. This altered concentration of bacteria was also commensurate with disease severity and researchers concluded that compositional changes play a role in exacerbating disease by contributing to dysregulation of the immune response. Several of the bacteria that were abundant in COVID-positive patients are associated with dysregulated inflammation in other illnesses. COVID-negative patients had an abundance of bacteria that are associated with reduced inflammation and better immune system regulation. It is important to note that these alterations in microbial flora persisted beyond recovery from COVID-19. The authors went on to state that microbiome analysis may help to determine individuals at risk of severe disease and the potential development of ongoing inflammation as seen in long COVID and may also be useful in guiding intervention with specific probiotics [11]. The authors of this study also state that it has not been determined if the microbial composition of COVID-positive patients occurred prior to or as a result of the infection. In another recently published article in Gut Pathogens, Chhibber-Goel focuses on the role of angiotensin-converting enzyme 2 (ACE2) and the ACE2 receptor that specifically applies to the infectivity of SARS-CoV-2. Chhibber-Goel states that the number of ACE2 receptors increases in the duodenum with age, which increases viral entry and replication. With increased binding of the ACE2 receptors, the production of ACE2 is reduced. In the gut, ACE2 is responsible for supporting the gut endothelium and the microbial communities within the gut by improving circulation. Impaired ACE2 expression is associated with viral infection, immune imbalance and bacterial dysbiosis in the intestines [12]. Dysbiosis of the gut modulates the immune response of neutrophils, T cell subsets, inflammatory cytokines, and TLRs, which have an influence on lung function and the ability to mount a balanced immune response [12]. As stated by Chhibber-Goel, the gut microbiome is changed during the COVID-19 infection and increases host susceptibility to other infections or potentially unmasks an underlying infection that was kept in check by a healthy microbiome and a balanced immune response. This shift in the microbiome, where there is a change in the type and volume of bacteria beyond the initial infection, has been implicated in the development of long COVID. Use of probiotics to support a healthy microbiome confers protection from infections through direct competition with disease-causing microbes, enhancement of epithelial barrier functions, and support of a balanced and robust immune response. In addition to the gut-lung axis, the more familiar gut-brain axis is also an important consideration in the development of long COVID. Gut microbiota exerts significant effects on the central nervous system (CNS), brain neurochemistry, and activity through microbial products such as short-chain fatty acids (SCFA) and serotonin. These microbial products influence brain immunity through signaling pathways or by directly crossing the blood-brain barrier [10]. In particular, SCFAs have a role in the development and function of microglia, which are the brain’s macrophages involved in antigen presentation, phagocytosis, and modulating inflammation. The CNS also affects the gut microbiota via secretion of catecholamines that further influences gut physiology and barrier integrity via autonomic nervous system function. Modulation of gut microbiota with probiotics has shown positive effects on neuroinflammatory disorders, anxiety, depression, and symptoms associated with chronic fatigue [10]. Supporting a healthy and balanced immune response by promoting an abundant and diverse microbiome may help reduce the severity of acute COVID, as well as the lasting sequelae of long COVID. Benefits of Reducing Viral Load Early There are definite benefits to reducing viral replication early in the disease process. As stated earlier, what occurs in the replication phase of COVID-19 determines what will occur later in the disease—and this may also be true for long COVID. The issue is and has been that there are no broadly accepted outpatient treatments for early COVID-19 that would inhibit viral replication within the first few days of diagnosis. When considering potential pharmaceuticals to treat early COVID, the best and quickest options are repurposed medications with known safety profiles and mechanisms of action that can be effectively applied to the pathophysiology of SARS-CoV-2. Some of the options that are currently being considered fall under the categories of antivirals, immune-modulating treatments, antithrombotics, and other medications with specific actions that can prevent replication of the SARS-CoV-2 virus [13]. As early interventions are implemented, the relationship between reduced viral replication during early infection and the potential reduction in the development of long COVID will become more defined as conclusions are drawn from the data. At present, research is more focused on the immediate outcomes of reducing disease severity, hospitalization, and death. Supporting a Healthy Immune Response Until the world of medicine can agree upon some form of early pharmaceutical treatment that is appropriate in the outpatient setting, is scalable to large populations, and is safe, supporting the immune system and general health through nutritional supplements, dietary, and lifestyle measures are the best and most readily available options. Following a plant-rich anti-inflammatory diet that is similar in macronutrient balance to the Mediterranean diet is a good starting point and is supportive of a robust and diverse microbiome. Adding in nutritional supplements that support the immune system with targeted nutrients to slow the spread of viruses is also key. Nutrients that are helpful include vitamin D, C, A, quercetin, fish oil, zinc, selenium, NAC, probiotics, and melatonin to name a few. A good night’s sleep and regular exercise are also key in supporting the immune system and reducing stress. In the next installment on long COVID, we will explore issues related to mitochondrial dysfunction and its relationship to post-viral syndrome. References 1. Acharya D, Liu G, Gack MU. Dysregulation of type I interferon responses in COVID-19. Nat Rev Immunol. 2020;20(7):397-398. 2. McCarty MF, DiNicolantonio JJ. Nutraceuticals have potential for boosting the type 1 interferon response to RNA viruses including influenza and coronavirus. Prog Cardiovasc Dis. 2020;63(3):383-385. 3. Yang H, Lyu Y, Hou F. SARS-CoV-2 infection and the antiviral innate immune response. J Mol Cell Biol. 2021;12(12):963-967. 4. Scientists trace severe COVID-19 to faulty genes and autoimmune condition. Science News. The Rockefeller University. https://www.rockefeller.edu/news/29183-severe-covid-19-faulty-genes-autoimmune-condition. Accessed March, 2021. 5. Lyons-Weiler J. Pathogenic priming likely contributes to serious and critical illness and mortality in COVID-19 via autoimmunity. J Transl Autoimmun. 2020;3:100051. 6. Vojdani A, Kharrazian D. Potential antigenic cross-reactivity between SARS-CoV-2 and human tissue with a possible link to an increase in autoimmune diseases. Clinl Immunol. 2020; 217:108480. 7. Mueller SN, Rouse BT. Immune responses to viruses. Clinical Immunology. 2008:421–431. 8. Fujinami RS, von Harrath MG, Christen U, et al. Molecular mimicry, bystander activation, or viral persistence: infections and autoimmune disease. Clin Microbiol Rev. 2006;19(1):80-94. 9. Oldstone MB. Viral persistence: parameters, mechanisms and future predictions. Virology. 2006;344(1):111-118. 10. Shahbazi R, Yasavoli-Sharahi H, Alsadi N, et al. Probiotics in treatment of viral respiratory infections and neuroinflammatory disorders. Molecules. 2020;25(21):4891. 11. Yeoh YK, Zuo T, Lui GCY, et al. Gut microbiota composition reflects disease severity and dysfunctional immune responses in patients with COVID-19. Gut. 2021;70(4):698-706. 12. Chhibber-Goel J, Gopinathan S, Sharma A. Interplay between severities of COVID-19 and the gut microbiome: implications of bacterial co-infections? Gut Pathog. 2021;13(1):14. 13. Kim PS, Read SW, Fauci AS. Therapy for early COVID-19: a critical need. JAMA. 2020;324(21):2149–2150.